CARBON DIOXIDE, NARCOSIS,
AND DIVING
BY JOHNNY E. BRIAN JR., M.D.
Carbon dioxide (CO2) is the gaseous end product of the aerobic
metabolism of oxygen. CO2 is highly soluble in body tissues, and
readily diffuses from cells to blood, where circulation transports
it to the lungs for elimination. Divers often ignore carbon
dioxide, as CO2 is a normal part of life. However, CO2 may have
definite and detrimental effects if a diver accumulates an
excessive amount of CO2. Understanding how CO2 can become elevated,
the symptoms, and the consequences of elevated CO2 can only make us
safer divers.
Air contains only 0.03% CO2; therefore, under normobaric
conditions, air inspired into the lungs is almost devoid of CO2.
This creates a large difference in the partial pressure of CO2
(PCO2) between blood and inspired air, promoting CO2 to diffuse
rapidly from blood into the gas phase of the lungs. At rest,
ventilation is controlled by the PCO2 in the ventilatory control
center of the brain. The nervous system adjusts ventilation to
maintain arterial blood PCO2 (PaCO2) constant, which at rest ranges
from 35-45 mmHg (average 40 mmHg). Venous blood entering the lungs
has a CO2 partial pressure (PvCO2) approximately 5 mmHg higher than
arterial blood, or 45 mmHg. Because CO2 is very soluble in blood, a
large volume of CO2 exists in a dissolved state in blood. This
means that to lower blood PCO2 any given amount, a large amount of
CO2 must be removed. As CO2 diffuses into the gas space (alveoli)
of the lungs, an equilibrium is established when the alveolar gas
phase partial pressure of CO2 (PaCO2) and blood PCO2 reach 40 mmHg.
The volume of gas breathed per minute (minute ventilation) controls
removal of CO2 from the blood perfusing the lungs. When CO2
production increases during exercise at 1 ATA, minute ventilation
also increases to maintain PaCO2 constant. With severe exercise at
1 ATA, PaCO2 may decrease slightly. During exercise, if minute
ventilation does not increase to match the increase in CO2
production, then arterial PCO2 will increase.
Carbon dioxide is a narcotic gas capable of depressing awareness
to the degree of total loss of consciousness. In humans, acute
elevation of arterial PCO2 above 70-75 mmHg reduces the level of
awareness (20), and PaCO2 above 100-120 mmHg produces
unresponsiveness (26). Severe elevation of PaCO2, by inhalation of
30%-40% CO2 (220-300 mmHg), produces surgical anesthesia in both
animals and humans (14,25). In dogs, an arterial PCO2 above 250
mmHg results in a state of general anesthesia (2). Carbon dioxide
has not been useful as a general anesthetic, as severe elevation of
PCO2 produces marked derangement in acid-base balance. In addition,
anesthetic levels of CO2 produce seizures in both animals and
humans (1, 14, 25).
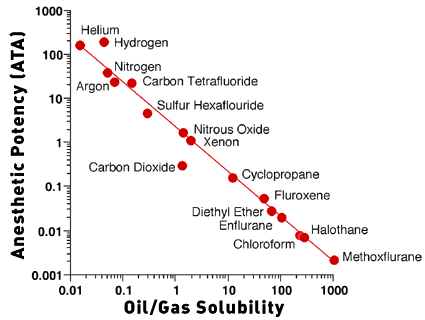
Carbon dioxide is 25 times more lipid-soluble than nitrogen, and
lipid solubility has been correlated with the narcotic potency of
gases. Figure 1 is a plot of oil/gas solubility versus anesthetic
potency of gases and inhaled anesthetics. These gases fall along
the line that indicates a high degree of correlation between lipid
solubility and anesthetic potency. Xenon and nitrous oxide have
approximately the same lipid solubility as CO2. If the anesthetic
effect of CO2 was produced only by lipid solubility, then the CO2
point should lie along the line with the nitrous oxide and xenon
points. CO2, however, falls below the line, which means that
anesthesia is produced by a lower partial pressure of CO2 than
would be predicted from the lipid solubility. The anesthetic
potency of CO2 is about 130 times that of nitrogen, much greater
than the ratio of lipid solubilities of CO2 and nitrogen. This
suggests that CO2 produces an anesthetic effect independent of
lipid solubility.
Elevation of CO2 has been associated with a decreased level of
consciousness during both hyperbaric chamber and wet dives. Case
and Haldane used inspired CO2 to elevate arterial PCO2 at 1 ATA,
and during hyperbaric exposures to 300 FSW, in human volunteers
(1). Most data reported in this study are subjective impressions;
therefore, the objective measurements are limited. However, the
paper is a fascinating report of early diving research, including a
description of spinal bends in Haldane after a He/O2 dive (1). At 1
ATA, 6%-8% (45-60 mmHg) inspired CO2 produced a marked increase in
respiration, but little change in mental or physical skills (1).
Although Case and Haldane did not measure arterial PCO2, it was
most likely less than 80 mmHg under the conditions at 1 ATA. The
exposures were then repeated after compression to 300 FSW. The
subjects noted that there was much less increase in ventilation
during inspired CO2 at 300 FSW. This suggests that the subjects
were unable to increase minute ventilation to an equal level as
during the 1 ATA exposure. One can only deduce that the increase in
arterial PCO2 was more severe at 300 FSW than at 1 ATA. At 300 FSW
during CO2 inspiration, there was severe impairment of both mental
and physical skills. Subjects noted that the "narcosis" was much
more severe than with exposure to air alone at 300 FSW. When
inspired CO2 was increased to a level of 0.8%-0.9% at 300 FSW
(which equals 8-9% at 1 ATA; 60-70 mmHg), subjects quickly lost
consciousness and some seized. Subjects were described as lapsing
into unconsciousness "quietly and easily" Although PCO2 was not
measured, arterial PCO2 was likely greater than 80 to 100 mmHg at
300 FSW. Case and Haldane theorized that the respiratory response
to CO2 was suppressed by nitrogen narcosis.
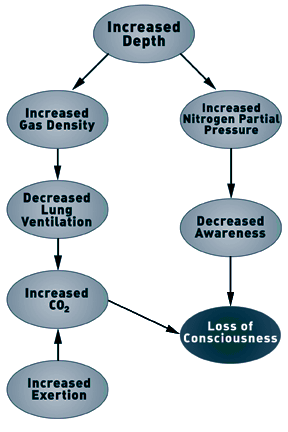
Warkander et al. studied CO2 accumulation during exercise at 6.8
ATA, and reported 2 subjects that required rescue from a wet pot
due to severe CO2-induced incapacitation (24). Both subjects had
elevation of arterial PCO2 above 80-90 mmHg, and both were unaware
of their incapacitation. In the same study, other subjects
continued to function with similar elevation of arterial PCO2. This
suggests that CO2-induced depression of awareness may vary greatly
between individuals.
Carbon dioxide reduces mental and physical capacity at
sub-anesthetic concentrations. Hesser et al. studied the effect of
increased CO2 in volunteers under normobaric and hyperbaric
conditions (6,7). They found that a modest increase of PCO2 to
50-60 mmHg significantly reduced the ability to perform mental
skills such as arithmetic and color naming, as well as physical
skills, such as manual dexterity and eye-hand coordination. They
concluded that the effect of CO2 was additive to, but not
synergistic with, nitrogen narcosis. Fothergill et al. also studied
the effect of PCO2 elevation to 50-60 mmHg on a battery of mental
tests in volunteers, reporting that the modest increase in PCO2
reduced the number of correct responses principally by reducing the
number of attempts at the tests (4). This suggests that increased
PCO2 slows comprehension of presented information. The data also
suggests that modest elevation of PCO2, which may occur during
diving, may contribute to "narcosis" independent of elevation of
PN2.
Although Case and Haldane theorized that narcosis limited the
respiratory response to CO2, it is the increase in gas density, and
not the narcotic properties of the gas, that limits the ventilatory
response under hyperbaric conditions (5,10). As the rate of lung
ventilation increases, exhaling becomes an active process, with
increased intrathoracic pressure (the pressure inside the chest)
increasing the rate of gas flow out of the lungs. Progressive
increase in the force of exhalation will increase the gas flow
rate, but only up to a point. The airways that conduct gas in and
out of the lungs can be compressed and collapsed by pressure on the
outside of the airways. The intrathoracic pressure is the pressure
applied to the outside of the airways. During forced exhalation,
intrathoracic pressure rapidly rises above the pressure at which
airways collapse. When the airways begin to collapse, the flow of
gas out of the lungs is obstructed. Thus, gas flow is slowed. The
maximal possible expiratory gas flow rate occurs when the airways
just begin to collapse. This means that the expiratory gas flow
rate cannot be increased beyond the point when airways begin to
collapse, regardless of how much effort is exerted. Exhalation is
frequently termed "effort independent", as forced expiratory effort
cannot overcome the expiratory obstruction due to airway
collapse.
Under normobaric and hyperbaric conditions, the single factor
that limits the ability to increase ventilation is the rate at
which gas can be exhaled from the lungs. The ability to exhale gas
is reduced during hyperbaric and diving conditions. As gas density
increases, increased effort is required to exhale gas (i.e.,it
takes more work to move a heavier gas). However, the amount of work
that can be generated (the pressure differential) is limited by the
collapse of the airways. Airways collapse at the same intrathoracic
pressure under normobaric and hyperbaric conditions. This means
that to exhale gas, the amount of work is fixed and equal under
normobaric and hyperbaric conditions. Moving denser gas with the
same amount of work means that airways begin to collapse at a lower
expiratory gas flow rate. The result is that the maximal possible
lung ventilation per minute is progressively reduced as gas density
increases.
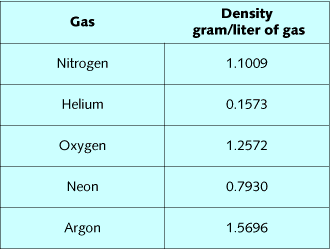
A common method to measure the respiratory response to CO2 is to
allow a subject to breathe CO2 and measure the increase in lung
ventilation. Nitrox at 4 ATA attenuates the increase in lung
ventilation with inspired CO2; reducing gas density with He/O2
restores the CO2 response to the 1 ATA baseline (10). Breathing air
at 4 ATA (99 FSW) reduces maximal expiratory gas flow rate and
maximal lung ventilation per minute to one-half that present at 1
ATA (27). The effect on lung ventilation is more marked at greater
ambient pressure or with gases of greater density. The ability to
increase ventilation and eliminate CO2 during exertion may be
significantly limited by increased gas density. Thus, maintenance
of a normal PaCO2 may not be possible when breathing dense gas.
Elevated CO2 is normally a potent respiratory stimulus and,
under normobaric conditions, causes increased respiratory rate
(hyperventilation) and the sensation of shortness of breath.
Further elevation of PCO2 leads to headache, dizziness, nausea, and
eventually a reduced level of consciousness. Similar symptoms occur
during diving and hyperbaric exposure, although some have reported
that the sensations of hyperventilation and shortness of breath may
not be noted (24). It is possible that, during diving, CO2-induced
dizziness could be mistaken for nitrogen narcosis. Although
increased CO2 is normally a potent respiratory stimulus, elevation
of PCO2 to levels associated with a decreased level of
consciousness (100- 200 mmHg or greater) progressively depresses
respiration (18, 19). Thus, severe elevation of PaCO2 will cause
further CO2 retention by reducing lung ventilation.
Gas density is a critical element in the respiratory response to
exertion at depth. Table 1 lists densities of diving gases, while
table 2 lists the composite densities of diving mixes. By summing
the fractional gas densities of a mix, and then multiplying it by
depth in ATA, the density of the mix can be calculated. Air at 99
FSW, 32% nitrox at 99 FSW, 16/55 at 200 FSW, and 10/70 at 300 FSW
all have approximately the same density. The effect of these mixes
on the ability to breathe and eliminate CO2 should be very similar.
Oxygen is slightly denser than nitrogen, so substitution of oxygen
for nitrogen slightly increases mix density relative to air.
At rest, while breathing nitrox at 4 ATA, PCO2 is normal,
indicating adequate ventilation to eliminate CO2 (10). During
exertion, however, the increase in lung ventilation is less than
occurs at 1 ATA, and PCO2 rises to a significantly higher level
than during exercise at 1 ATA (5,10). When lung ventilation
approached the maximum possible at a given gas density, PCO2 must
increase. The response of ventilation and PCO2 to exercising while
breathing dense gas has been tested a number of times
(3,10,11,22,27). These studies were directed more at commercial
diving conditions, with short periods of exercise (minutes) and
high levels of exertion. In addition, these studies were conducted
under optimal respiratory conditions, with subjects breathing from
very low resistance gas circuits. Because of the conditions of
these studies, they are less helpful in determining allowable
exercise levels in technical and cave diving, where exertion is
less but over a longer period of time. In addition, in-water divers
breathe from demand valve regulators, which may impose additional
work when breathing.
When breathing air under optimal respiratory conditions at 4 ATA
(or gas of equivalent density), the maximal possible ventilation
for a very short time period (maximum voluntary ventilation) is 3
to 3.5 ft3/min (10,27). During exertion, lung ventilation can
usually be sustained at 75% of the maximal voluntary ventilation
(15), which would translate into 2.7 ft3/min with a low resistance
breathing circuit. Real-life in-water diving is usually conducted
under less than optimal conditions. It should therefore be expected
that the maximal possible minute ventilation would be less. Divers
swimming at 50-60 ft/min require a ventilation rate of about 0.6
ft3/min or less (13). Experience indicates that breathing less than
1 ft3/min of gas during technical and cave diving is tolerated
without symptoms of CO2 accumulation. However, as gas consumption
increases above 1 ft3/min, especially as gas consumption approaches
2 ft3/min, there is increased likelihood of CO2 accumulation and
resultant deleterious effects.
A number of studies have reported that divers have an abnormal
respiratory response to CO2 (8,9,12,17). Lanphier reported that US
Navy divers swimming at about 75 ft/min exhibited abnormal
elevation of PCO2 that averaged 55 mmHg (12,13). The study-subjects
were hardhat divers, in whom inadequate helmet ventilation often
causes CO2 rebreathing. These divers were later exercised at 1 ATA,
where they also exhibited marked and abnormal elevation of PCO2
(12). Lanphier theorized that chronic CO2 rebreathing in these
divers led to CO2 insensitivity. However, Kerem et al. studied open
circuit scuba divers, and also reported a reduction of the
respiratory response to CO2; (8)Sherman et al. (21) reported
similar findings. These findings suggest that chronic CO2
rebreathing is not required for a diver to develop a depressed
respiratory response to CO2. The depressed respiratory response to
elevation of CO2 appears to vary greatly between individuals, with
some divers being normal and other having a very depressed CO2
response (12, 16). Divers may consciously reduce their rate of
ventilation to conserve gas, which would lead to CO2 accumulation.
Because most diving mixes are relatively hyperoxic, hypoxia with
reduced ventilation is unlikely. Lanphier et al. attempted to
develop a normobaric screening test to identify individuals with
reduced respiratory response to CO2. Unfortunately, only testing
under hyperbaric conditions was successful (13). The existence and
prevalence of impaired CO2 response in cave and technical divers is
not known.
Scuba regulators can add additional resistance to breathing,
limiting the ability to eliminate CO2. Almost all studies of
respiratory dynamics at depth are conducted under optimal
respiratory conditions. There has been very limited study of the
effect of demand valve regulators on the ability to breathe at
depth. Breathing air at 4 ATA significantly reduces the maximum
possible minute ventilation; moreover, the addition of a demand
valve regulator causes a very slight additional reduction in
ventilation (23). Increasing the resistance of breathing at 4 ATA
causes a slight increase in PCO2 during He/O2 breathing (12).
However, Lanphier reported that during exertion and air breathing
at 7.8 ATA, restriction of breathing rapidly resulted in
unconsciousness, most likely due to CO2 retention (12). The overall
impact of breathing through a modern, well-maintained scuba
regulator on the response of PCO2 to exercise is unknown.
Notwithstanding, breathing resistance should be kept to a minimum
to reduce the possibility of CO2 retention.
The primary cause for CO2 elevation during diving, then, is
exertion coupled with increased gas density. Stress increases the
metabolic rate and can contribute to increased CO2 production.
Rebreathing expired gas containing CO2 will also elevate PCO2.
However, significant rebreathing seems unlikely with standard
demand-valve scuba regulators, as they have minimal dead space.
Devices with increased dead space, such as communication systems
and full-face masks, may elevate CO2 by rebreathing. Rebreathers
can also elevate CO2 due to malfunction of the one-way valves or
exhaustion of the CO2 absorbent. The ability to perform exertion at
1 ATA should not be used as a guide for exertion at depth, as the
ability to ventilate the lungs may be significantly limited by the
increased gas density. Divers should monitor themselves and their
buddies for signs and symptoms of elevated PCO2. Increased CO2
impairs mental and physical skills and may hamper self-rescue.
Severe elevation of CO2 can depress the level of awareness and
prevent a diver from recognizing and reversing the process. Divers
have become incapacitated and lost consciousness due to CO2
retention without being aware of being in a life-threatening
situation. Elevated CO2 also increases the likelihood of hyperoxic
seizures.
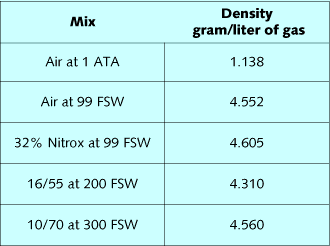
If a diver experiences symptoms of elevated CO2, they should
stop their exertion and relax, if possible. This will reduce CO2
production, and should allow time for the ventilation to eliminate
the excess CO2. If this is not possible, then the dive should be
terminated. Ascent to a shallower depth will be beneficial by
reducing gas density and allowing more effective ventilation to
eliminate CO2. Incapacitated but breathing divers should also be
taken to a shallower depth for the same reason. Elimination of
excess CO2 and recovery of consciousness may be possible once gas
density is reduced.
REFERENCES
1. Case, E. M., and J. B. S. Haldane. Human physiology under
high-pressure I. effects of nitrogen, carbon dioxide and cold. J.
Hyg. 41: 225-249, 1941.
2. Eisele, J. H., E. I. Eger, II, and M. Muallem. Narcotic
properties of carbon dioxide in the dog. Anesthesiology 28:
856-865, 1967.
3. Fagraeus, L. Maximal work performance at raised air and
helium-oxygen pressures. Acta Physiol. Scand. 91: 545-556,
1974.
4. Fothergill, D. M., D. Hedges, and J. B. Morrison. Effects of
CO2 and N2 partial pressure on cognitive and psychomotor
performance. Undersea Biomed. Res. 18: 1-19, 1991.
5. Gelfand, R., C. J. Lambertsen, and R. E. Peterson. Human
respiratory control at high ambient pressures and inspired gas
densities. J. Appl. Physiol. 48: 528-539, 1980.
6. Hesser, C. M., J. Adolfson, and L. Fagraeus. Role of CO2 in
compressed-air narcosis. Aerosp. Med. 42: 163-168, 1971.
7. Hesser, C. M., L. Fagraeus, and J. Adolfson. Roles of
nitrogen, oxygen, and carbon dioxide in compressed-air narcosis.
Undersea Biomed. Res. 5: 391-400, 1978.
8. Kerem, D., Y. Melamed, and A. Moran. Alveolar PCO2 during
rest and exercise in divers and non-divers breathing O2 at 1 ATA.
Undersea Biomed. Res. 7: 17-26, 1980.
9. Lally, D. A., F. W. Zechman, and R. A. Tracy. Ventilatory
responses to exercise in divers and non-divers. Respir. Physiol.
20: 117-129, 1974.
10. Lambertsen, C. J., R. Gelfand, M. J. Lever, G. Bodammer, N.
Takano, T. A. Reed, J. G. Dickson, and P. T. Watson. Respiration
and gas exchange during a 14-day continuous exposure to 5.2% O2 in
N2 at pressure equivalent to 100 FSW (4 ATA). Aerosp. Med. 44:
844-849, 1973.
11. Lambertsen, C. J., R. Gelfand, R. Peterson, R. Strauss, W.
B. Wright, J. G. Dickson, Jr., C. Puglia, and R. W. Hamilton, Jr.
Human tolerance to He, Ne, and N2 at respiratory gas densities
equivalent to He-O2 breathing at depths to 1200, 2000, 3000, 4000,
and 5000 feet of sea water (predictive studies III). Aviat. Space
Environ. Med. 48: 843-855, 1977.
12. Lanphier, E. H. Influence of increased ambient pressure upon
alveolar ventilation. In:C. J. Lambertsen and L. J. Greenbaum, Jr.
Second Symposium on Underwater Physiology. Washington, D.C.:
National Academy of Sciences, 1963: 124-133.
13. Lanphier, E. H., and E. M. Camporesi: Respiration and
exertion In P. B. Bennett and D. H. Elliott (eds): The Physiology
and Medicine of Diving. London, W. B. Saunders Company Ltd., 1993,
77-120
14. Leake, C. D., and R. M. Waters. The anesthetic properties of
carbon dioxide. J. Pharmacol. Exp. Ther. 33: 280-281, 1928.
15. McArdle, W. D., F. I. Katch, and V. L. Katch: Exercise
Physiology. Baltimore, Williams and Wilkins, p. 259, 1996.
16. Morrison, J. B., J. T. Florio, and W. S. Butt. Observations
after loss of consciousness under water. Undersea Biomed. Res. 5:
179-187, 1978.
17. Morrison, J. B., J. T. Florio, and W. S. Butt. Effects of
CO2 insensitivity and respiratory pattern on respiration in divers.
Undersea Biomed. Res. 8: 209-217, 1981.
18. Nunn, J. F.: NunnÕs Applied Respiratory Physiology.
Oxford, Butterworth-Heinemann, Ltd., 1993, pp 90-114.
19. Raymond, L. W., and F. G. Standaert. The respiratory effects
of carbon dioxide in the cat. Anesthesiology 28: 974-980, 1967.
20. Refsum, H. E. Relationship between state of consciousness
and arterial hypoxaemia and hypercapnia in patients with pulmonary
insufficiency, breathing air. Clin. Sci. 25: 361-367, 1963.
21. Sherman, D., E. Eilender, A. Shefer, and D. Derem.
Ventilatory and occlusion-pressure responses to hypercapnia in
divers and non-divers. Undersea Biomed. Res. 7: 61-74, 1980.
22. Varene, P., H. Vieillefond, C. Lemaire, and G. Saumon.
Expiratory flow volume curves and ventilation limitation of
muscular exercise at depth. Aerosp. Med. 45: 161-166, 1974.
23. Vorosmarti, J., Jr. Influence of increased gas density and
external resistance on maximum expiratory flow. Undersea Biomed.
Res. 6: 339-346, 1979.
24. Warkander, D. E., W. T. Norfleet, G. K. Nagasawa, and C. E.
G. Lundgren. CO2 retention with minimal symptoms but severe
dysfunction during wet simulated dives to 6.8 ATA abs. Undersea
Biomed. Res. 17: 515-523, 1990.
25. Waters, R. M. Toxic effects of carbon dioxide. New Orleans
Med. Surg. J. 90: 219-224, 1937.
26. Westlake, E. K., T. Simpson, and M. Kaye. Carbon dioxide
narcosis in emphysema. Q. J. Med. 24: 155-173, 1955.
27. Wood, L. D. H., and A. C. Bryan. Exercise ventilatory
mechanics at increased ambient pressure. J. Appl. Physiol. 44:
231-237, 1978.
Copyright ©2004 Global
Underwater Explorers.
All rights reserved.
|